Project

What are we trying to achieve in this project?
Our main aim in this project is to advance the capabilities of time-domain fluorescence lifetime imaging (tdFLI) for real-time and non-invasive assessment of novel (patho-)physiological and molecular processes. Compared to traditional fluorescent imaging, tdFLI offers enhanced contrast, enabling better differentiation between real fluorescence and background signals. It also facilitates objective in situ planning of resection lines with potential reductions in surgical margins, improved detection of small malignancies at greater depths, sensitive identification of invaded lymph nodes, and accurate colocalization with vital organs (multiplexing). tdFLI can be used for endoscopic or intravascular procedures, whether with or without exogenously administered contrast agents (label-free), to improve the diagnosis, staging, and in situ characterization of cancerous tissue or suspicious lesions like tumors or atherosclerotic lesions.
One particularly promising aspect of tdFLI is its ability to monitor real-time parameters such as pH, tissue oxygenation, and other metabolites. This novel approach holds great potential for assessing tissue viability during surgical interventions. Prompt recognition of decreased tissue oxygenation can facilitate immediate reestablishment of blood flow and help predict tissue desaturation-related injuries. Moreover, tdFLI can assist in the precise excision of devitalized tissues. Furthermore, by enabling accurate and reliable real-time measurements, tdFLI allows for monitoring dynamic changes deep within wound environments, such as those resulting from conditions like diabetes or burns.
We have developed the following research objectives:
- RO1. Develop the technological foundations that are needed to enable real-time in vivo tdFLI in the near-infrared region (NIR): time-domain NIR image sensors, biocompatible fluorescent contrast agents with programmable FLTs and time-domain models, methods and algorithms that can extract and objectify the hidden biomedical information.
- RO2. Integrate these foundations into an imaging system and provide the seeds for a technical ecosystem in which such a system can exist, software, validation techniques and characterization tools such as phantoms.
- RO3. Demonstrate and disseminate the capabilities and potentials of this novel technology through in vivo proof-of-concepts with the goal to facilitate technology acceptance by end-users in the biomedical field and uptake in new markets and applications.
These research objectives requires novel developments from widely different fields:
- Semiconductor physics and circuits: novel fast highly-efficient time-domain image sensors
- Photonic engineering: development of a fluorescence imaging instrument with fast-pulsed and spatially modulated illumination
- Mathematics: modelling of time-dependent light transport in tissue
- Computer science and artificial intelligence (AI): implementing real-time methods for processing and displaying the time-domain data
- Bio-chemistry: the creation of novel and smart biocompatible time-domain optimized fluorescent dyes
- Biology: design and execution of intricate experiments to validate the power of tdFLI.
In essence, our goal is to bring together the various developments in an iterative and novel manner, with the anticipation of achieving a revolutionary breakthrough in the field of optical imaging.
Our project involves two development cycles to achieve the final proof-of-concepts (PoCs) for medical applications. It is divided into three major phases: assessment, adjustment, and breakthrough.
During the assessment phase, we will initiate the development of core technologies based on our assumptions. We will explore the possibilities and limitations of our initial system using various in vitro and in vivo models. This phase will provide valuable insights into the possibilities and limitations of our initial system.
In the adjustment phase, we will compile the results from the assessment phase and use them to guide the refinement of our technology development. By aligning our efforts with the right targets, we can ensure that our project progresses in the most effective direction.
In the breakthrough phase, all developments come together, are finetuned and demonstrated in proof-of-concepts.
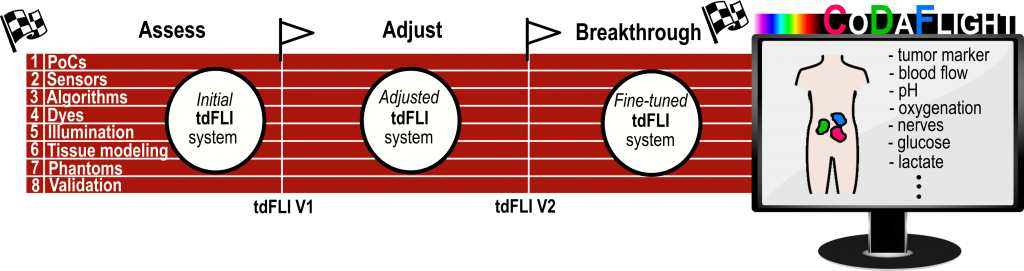
To facilitate our project’s execution, we have organized the work packages (WPs). WPs 1 to 3 correspond to the research objectives (RO1 to RO3) we have defined. Additionally, we have incorporated WP4, which focuses on communication, dissemination, and exploitation of our findings. WP5 is dedicated to project management, ensuring smooth coordination and oversight throughout the project.
Within central WP1, our focus is on studying and developing the necessary technological enablers for tdFLI. VUB-ETRO will take charge of designing fast current-assisted sensors incorporating microlens light steering. These sensors aim to achieve high quantum efficiency, fast detection, and small pixel sizes. Leveraging the extensive expertise of UoB, we will design novel biocompatible fluorescent dyes that contain pyrrole units and undergo structural modulation. The goal is to tune their fluorescence lifetime properties to align with the specific medical requirements. The FORTH team will contribute their expertise in tissue light transport modeling to investigate the time-dependent behavior of near-infrared (NIR) fluorescence in complex tissue. Their research will involve devising appropriate illumination techniques and methods for time-gated detection, as well as accurate reconstructions of the acquired data. Subsequently, UMG will employ newly developed real-time smart algorithms to implement these methods effectively. A dedicated task within our project is to coordinate the different technological developments, ensuring their compatibility and seamless integration. We will focus on adapting these developments to one another to achieve optimized overall performance. By coordinating these efforts, we aim to bring about a cohesive and highly efficient system for tdFLI.
In WP2, we build a practical tdFLI instrument platform which can be used to experiment with the different illuminations, sensors and algorithms. The instrument will also be made compatible for endoscopes.
In WP3, we will provide the experimental support, both to verify our assumptions and to validate the resulting system. In several proof-of-concepts we will investigate and demonstrate the power of tdFLI for clinically relevant applications. FORTH will also employ its expertise in the production of realistic geometry specific tissue mimicking phantoms to assess tdFLI in a controlled setting. VUB-ICMI will provide its expertise in in vivo fluorescence imaging and assist VUB-ETRO in developing novel validation procedures and techniques for tdFLI and employ these strategies on our instrument using the novel dyes designed by UBFC and the phantoms developed by FORTH. UiB and UMG’s expertise in preclinical imaging and their access to translational animal models will enable the generation of validated proof-of-concepts on the use and advantages of tdFLI for different medical applications. Iterative interactions between all partners will lead to further refinements in the technological components and in the design and features of the imaging instruments as well as to the optimisation of the new developed fluorescent probes. Out of all the possibilities for tdFLI, we have chosen to demonstrate three different proof-of-concepts which we deem relevant for current and future medical needs, and which are well aligned with the expertise and capabilities of UiB, UMG and VUB-ICMI.
Techology and state-of-the-art
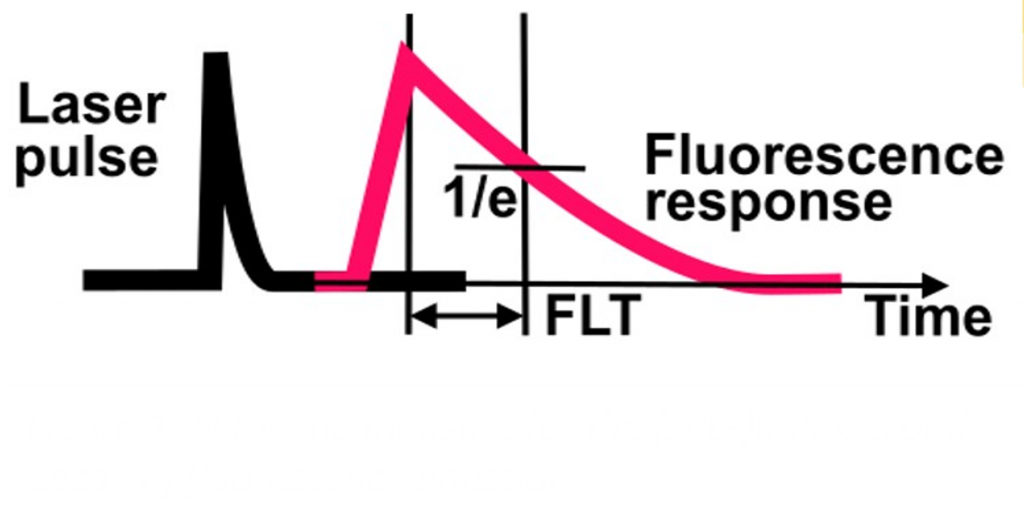
Conventional fluorescence imaging relies on the principle of exciting a fluorescent contrast agent with an appropriate light source and detecting the emitted photons with a specialized camera. Alternatively, one could measure the FLT, i.e. the average time a fluorophore remains in its excited state, either in the time-domain by recording the decay time of the fluorescence emission in response to pulsed illumination (Figure 1), or in the frequency-domain by measuring the phase shift of the fluorescence emission to a continuously modulated illumination. The current gold standard is Time-Correlated Single-Photon Counting (TCSPC), a time-domain technique built around a single-photon detector and counting electronics.
Alternatively, intensified CCDs offer a camera solution which can capture FLT images faster, without scanning. More recently, dedicated FLT cameras have been developed, based on novel image sensors such as frequency-modulated and time-gated image sensors. The recent advance of single-photon avalanche diode (SPAD) imagers also offers new, interesting possibilities for tdFLI. These novel technologies address many of the current limitations and are quite suited for microscopy and visible light applications. However, none of the above technologies are adapted to the needs of medical imaging because they have extremely poor quantum efficiencies (QE) in the so-called ‘tissue optical window’. Indeed, for in vivo applications, NIR wavelengths in the range of 700-800 nm are preferred because of lower autofluorescence, deeper tissue penetration (several mm to a cm) and reduced light scattering. Furthermore, NIR light is notoriously difficult to detect because NIR photons have very low energy. Additionally, currently available NIR dyes for clinical use exhibit very short, (sub-)ns FLT’s which require very fast sensors. Real-time NIR tdFLI imaging thus requires novel, more sensitive, and fast cameras.
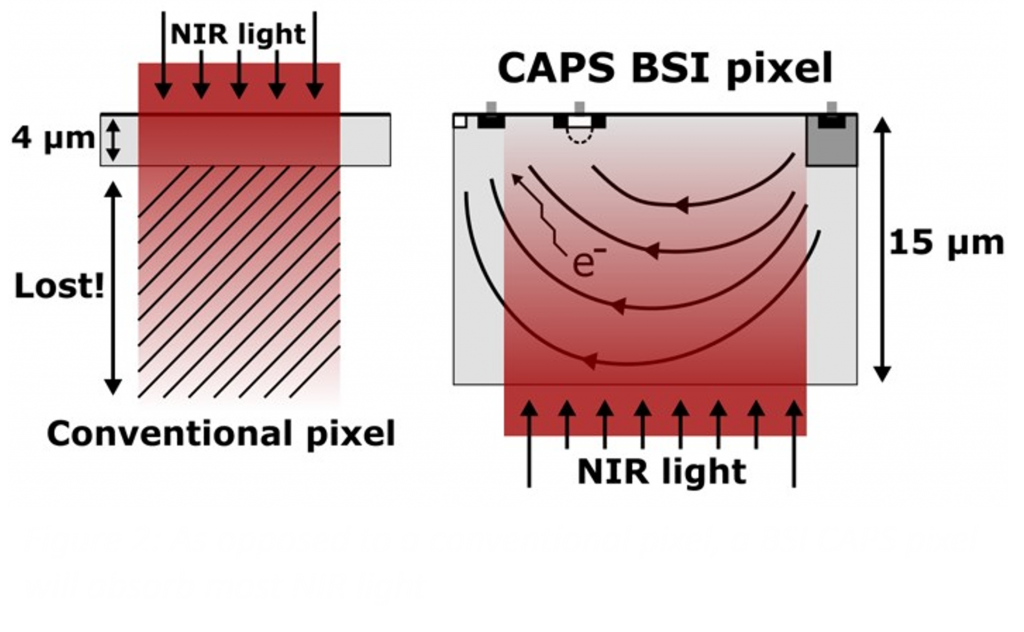
The Current Assisted Photonic Sampler (CAPS) image sensors of the VUB ETRO team have been conceived specifically for in vivo FLT imaging and to break through current NIR QE limitations. Current-assisted image sensors can have high NIR QE because they can be produced in a thick wafer substrate that allows absorbing nearly all NIR photons and use an electric field to accelerate photon-generated electrons to maintain high gating speed (Figure 2).
The same team have recently demonstrated that its current-assistance technology can also be applied to single-photon avalanche detectors (SPAD)offering an additional path towards high NIR QE FLT. While initially used to steer light into the small sensitive area of micrometer-sized pixels in mobile phones, new fabrication techniques have enabled microlenses for specialty pixels in e.g. SPAD or space image sensors. By combining microlenses with current-assistance in CoDaFlight, it will be possible to design a novel pixel that maximizes both QE and speed by directing both the light and the photo-generated charges.
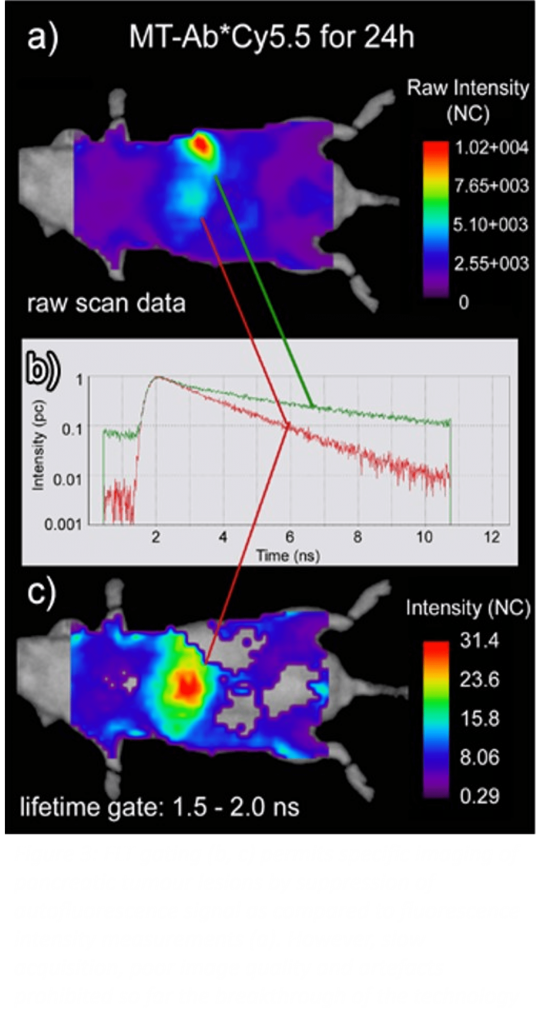
In basic research microscopy, FLT imaging has proven its power for decades for the accurate measurement of for instance ion concentrations, molecular binding or energy transfer interactions in in vitro configurations. However, thus far the medical applications of FLT imaging remain limited. UiB and UMG have demonstrated the high specificity of in vivo FLT imaging for decoupling exclusively specific signals of targeted tracers from autofluorescence and non-specific uptake (Figure 3). This results in improved imaging specificity and lower false-positive rates for FLT imaging over conventional fluorescence imaging. Furthermore, smart and nanoparticle-based probes have been applied preclinically for example for in vivo pH sensing, and lifetime-based photoluminescent oxygen sensing. However, as mentioned above, the imaging systems utilized are limited to the detection of FLTs in the visible wavelengths for which penetration depths of maximally a few 100 µm can be attained, and/or are operating on a slow point-based scanning approach to allow the detection of sufficient NIR photons. Therefore, clinical applications are very limited.
The methods and algorithms devised to extract FLT images from camera systems, such as the phasor approach, have been mostly inspired by microscopy applications and are insufficient for the different and challenging conditions of tissue imaging. For macroscopic applications, several attempts have been made to combine time-resolved approaches with appropriate light transport modelling and modern sensing and analysis methods, but only as a proof of concept and limited to the use of existing markers and imaging technologies with significant limitations in applicability. The CoDaFlight partner at FORTH recently made advantages in structured illumination and we intent to build on this in this project.
While fluorescent dyes that can sense molecular interactions, metabolites and other environmental parameters are widely available for FLT microscopy purposes, the number of FLT imaging-dedicated dyes for in vivo purposes is much more limited. Lanthanides, quantum dots and silicon nanocrystals have been investigated because of their unique FLT-properties, but they are generally associated with toxicity, poor pharmacokinetic profiles and/or non-specific accumulation in tissues, restraining their clinical use. The class of fluorescent organic dyes that is most often used for conventional fluorescence imaging in clinical practice and translational studies are cyanine-based organic dyes (ICG, IRdye800CW). However, the FLT of this class of NIR dyes is very short, typically 1 ns or less. This complicates differentiation from autofluorescence signals, which exhibit lifetimes ranging from 0.1 to a few ns. UBFC has extensive expertise in the synthesis and evaluation of fluorescent organic compounds for in vivo molecular imaging applications. These are particularly attractive for CoDaFlight because they exhibit unusually long FLTs for organic dyes (typically from 2 to 5 ns). Moreover, they offer a broad panoply of chemical strategies for their structural modification, allowing to finely tune their photophysical properties and water solubility, and to append further functional groups either for bioconjugation purpose or for making them responsive to their environment and relevant biostimuli.